Ramsey (1928), followed much later by Cass (1965) and Koopmans (1965), formulated the canonical model of optimal growth for an economy with exogenous ‘labour augmenting technological progress. The R.C.K model (or called. Ramsey (Neo-classical model) can be considered as an extension of the Solow model but without an assumption of a constant exogenous saving rate.
Assumptions
Firms
- Identical Firms.
- Markets, factors markets and outputs markets, are competitive.
- Profits distributed to households.
- Production fucntion with labour augmented techonological progress, \(Y=F(K,AL)\). (Three properties of the production: 1. CRTS; 2. Diminishing Outputs, second derivative<0; 3. Inada Condition.)
- \(A\) is same as in Solow model, \(\frac{\dot{A}}{A}=g\). Techonology grows at an exogenous rate “g”.
Households
- Identical households.
- Number of households grows at “n”.
- Households supply labour, supply capital (borrowed by firms).
- The initial capital holdings is \(\frac{K(0)}{H}\)., where \(K(0)\) is the initial capital, and \(H\) is the initial number of households.
- Assume no depreciation of capital.
- Households maximise their lifetime utility.
- The utility fucntion is constant-relative-risk-aversion (CRRA).
The lifetime utility for a certain household is represented by,
$$ U=\int_{t=0}^{\infty} e^{-\rho t}\cdot u(C(t))\cdot\frac{L(t)}{H} dt$$
Behaviours
Firms
By CRTS, \(\frac{\partial F(K,AL)}{\partial K}=\frac{\partial f(k)}{\partial k}\).
$$ F(K,AL)=AL\cdot F(\frac{K}{AL},1) \quad \text{By CRTS} $$
$$ \frac{\partial F(K,AL)}{\partial K}= AL \cdot \frac{\partial F(K/AL,1)}{\partial K}\cdot \frac{\partial K/AL}{\partial K}=F'(K/AL,1)\\=f'(k) $$
For the firms’ problem, the market is competitive, firms maximise their profits, then we get capital earns its marginal products,
$$ r(t)=\frac{\partial F(K,AL))}{\partial K}=f'(k(t)) $$
Similarly, labours earns its marginal products,
$$ W(t)=\frac{\partial F(K,AL)}{\partial L}=\frac{\partial F(K,AL)}{\partial AL}\cdot \frac{\partial AL}{L} $$
$$ =A \frac{\partial F(K,AL)}{\partial AL} =A \frac{\partial ALF(K/AL,1)}{\partial AL} $$
Apply the chain rule and replace \(F(K/AL,1)=f(k)\) and \(F'(K/AL,1)=f'(k)\). Then, we would get,
$$ W(t)=A[f(k)-kf'(k)]\ or\ W(t)=A(t)[f(k(t))-k(t)f'(k(t))]$$
We denote \(w(t)=W(t)/A(t)\) as the efficient wage rate, then we get,
$$ w(t)=f(k(t))-k(t)f'(k(t)) $$
Another key assumption of this model is,
$$ \dot{k}(t)=f(k(t))-c(t)-(n+g)k(t) $$
, which represents the actual investment (outputs minus consumptions), \(f(k(t))-c(t)\); and break-even investment, \((n+g)k(t)\). The implication is that population growth and technology progress would dilute the capital per efficient work.
The difference with the Solow model is that we do not assume constant saving rate “s” in \(sf(k)\) now, instead we assume the investment as \(f(k)-c\).
Households
The budget constraint of households is that: the PV of lifetime consumption cannot exceed the initial wealth and the lifetime labour incomes.
\int_{t=0}^{\infty} e^{-R(t)}\cdot C(t) \cdot \frac{L(t)}{H} dt \leq \frac{K(0)}{H}+\int_{t=0}^{\infty} e^{-R(t)}\cdot W(t) \cdot \frac{L(t)}{H} dt
, where \(R(t):=\int_{\tau=0}^ t r(\tau) d\tau\) to represent the discount rate overtime. When \(r\) is a constant, \(R(t)=r\cdot t\) and \(A(t)=A(0)e^{rt}=A(0)e^{R(t)}\).
The budget constraint can be rewritten as,
$$ \frac{K(0)}{H}+\int_{t=0}^{\infty} e^{-R(t)}\cdot[W(t)-C(t)]\cdot \frac{L(t)}{H} dt \geq 0 $$
And easy to know,
$$ \lim_{s\rightarrow \infty} [ \frac{K(0)}{H}+\int_{t=0}^{s} e^{-R(t)}\cdot[W(t)-C(t)]\cdot \frac{L(t)}{H} dt ] $$
$$ e^{-R(s)}\frac{K(s)}{H}= \frac{K(0)}{H}+\int_{t=0}^{\infty} e^{-R(t)}\cdot[W(t)-C(t)]\cdot \frac{L(t)}{H} dt $$
We get the non-Ponzi condition,
$$ \lim_{s\rightarrow \infty} \frac{K(s)}{H}\geq 0 $$
Then,
$$ \frac{K(s)}{H}=e^{R(s)}[ \frac{K(0)}{H}+\int_{t=0}^{\infty} e^{-R(t)}\cdot[W(t)-C(t)]\cdot \frac{L(t)}{H} dt] $$
Maximisation of Households’ Problem
We plug the \( \begin{cases} C(t)=A(t)c(t)\\L(t)=L(0)e^{nt}\\A(t)=A(0)e^{gt} \end{cases} \) into households’ lifetime utility function (objective function), and then get the,
$$ U=B\cdot \int_{t=0}^{\infty} e^{-\beta t} \frac{c(t)^{1-\theta}}{1-\theta} dt $$
, where \(B:=A(0)^{1-\theta} \frac{L(0)}{H}\) and \(\beta:=\rho-n-(1-\theta)g\) (we need \(\beta>0\) to make the utility function convergence), and the utility function is, \(u(c_t)=\frac{c_t^{1-\theta}}{1-\theta}\).
The budget constraint is the households’ lifetime budget constraints divided by \(A(0)\) and \(L(0)\),
$$ k(0)+\int_{t=0}^{\infty} e^{-R(t)}\cdot[w(t)-c(t)]\cdot e^{(g+n)t} dt \geq 0 $$
Lagrangian
$$ \mathcal{L}=B\cdot \int_{t=0}^{\infty} e^{-\beta t}\frac{c_t^{1-\theta}}{1-\theta} dt\\ +\lambda [k_0+\int_{t=0}^{\infty} e^{-R(t)}(w_t-c_t) e^{(n+g)t}dt ]$$
F.O.C.
$$B \cdot e^{-\beta t} c_t^{-\theta}=\lambda \cdot e^{-R(t)+(n+g)t} $$
Take logritham,
$$ ln(B)-\beta t-\theta ln(c_t)=ln(\lambda)-R(t)+(n+g)t $$
$$ ln(B)-\beta t-\theta ln(c_t)=ln(\lambda)-\int_{\tau=0}^t r(\tau) d\tau+(n+g)t $$
Thus, we get the relationship between consumption, \(c_t\), and \(R(t)\). Later, we take differentiation w.r.t. \(t\), and we would get,
$$ -\beta -\theta \frac{\dot{c_t}}{c_t} =-r(t)+n+g$$
So, (by replacing in the \(\beta\))
$$ \frac{\dot{c_t}}{c_t}=\frac{r(t)-n-g-\beta}{\theta}=\frac{r(t)-\rho-\theta g}{\theta}$$
Therefore, we get the time-path of consumption.
Dynamics
The dynamics of consumption
Combine the firms’ problems and households’ problems.
$$ \begin{cases} \frac{\dot{c_t}}{c_t}=\frac{r(t)-\rho-\theta g}{\theta} \\ r(t)=f'(k(t)) \end{cases} \Rightarrow \frac{\dot{c_t}}{c_t}=\frac{ f'(k(t)) -\rho-\theta g}{\theta} $$
Therefore, we find the time-path of consumption depends on \(f'(k)\). We define \(k*\) is the solution when \(f'(k)=\rho+\theta g\). So, at \(k^*\), the numerator of RHS equals zero.
As \(f(k)\) is an increasing function but with diminishing returns, so \(f”(k)<0\) and that means \(f'(k)\) is a decreasing function in k. Thus,
- at \(k<k^*\), \(f(k)>\rho+\theta g\) and \( \frac{\dot{c_t}}{c_t} >0\);
- at \(k>k^*\), \(f(k)<\rho+\theta g\) and \( \frac{\dot{c_t}}{c_t} <0\).
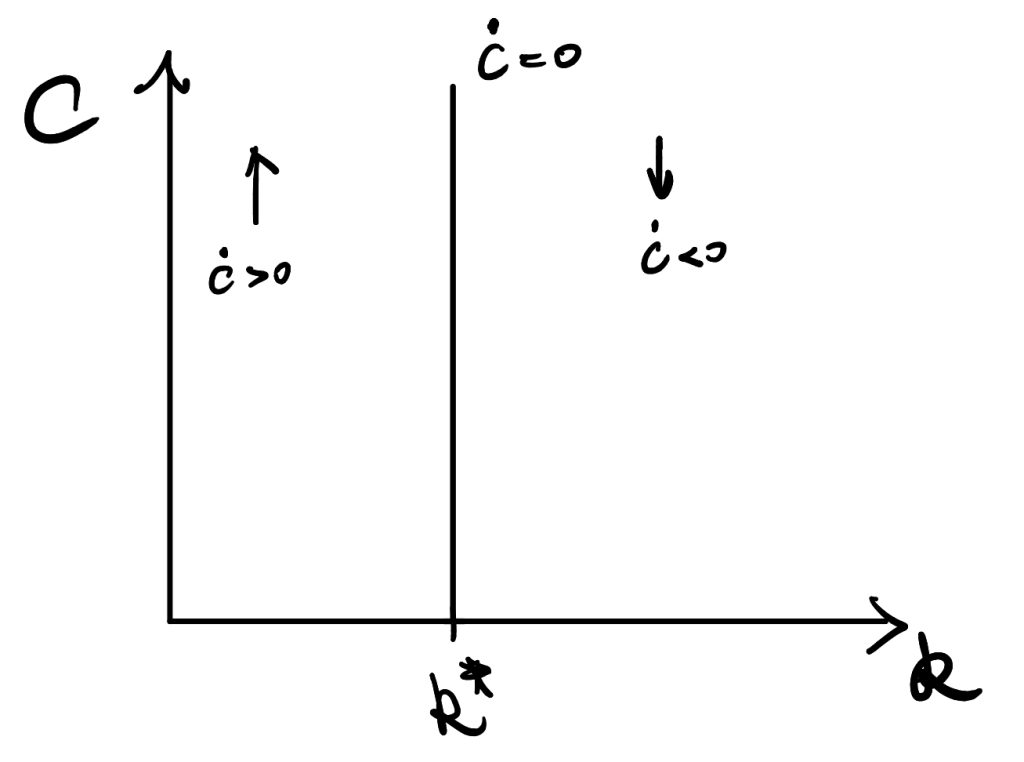
The dynamics of k
We recall the assumption,
$$ \dot{k}(t)=f(k(t))-c(t)-(n+g)k(t) $$
At \(\dot{k}=0\), consumption, \(c(t)=f(k(t))-(n+g)k(t)\), equals outputs minus break-even investment.
We now consider the Solow model without the depreciation term. Recall the difference between the RCK model and the Solow model is that we do not assume a constant saving rate over time, but other things keep similar. Thus, the term \(c(t)\) is “equivalent” to \(sy\) in Solow model.
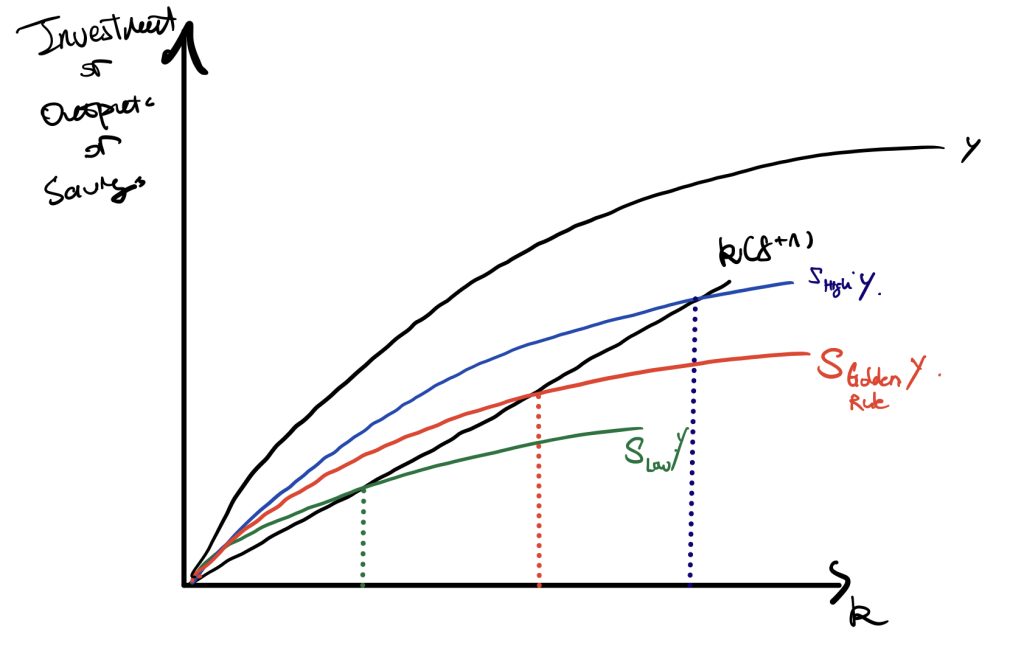
In the Solow model, changes in saving rate would change the magnitude of \(sy\) curve. The Golden Rule saving rate is “s” that maximises consumption (the difference between Y and the interaction between \(sy\) and \(k(g+n)\)). The shape of the production function determines the property of the Golden Rule saving rate.
An equilibrium level of consumption is determined in mainly two steps. 1. the interaction between saving \(sy\) and \((n+g)k\) determines the \(k^*\). 2. plug \(k^*\) back to \(sy\) and find the difference between outputs and savings to get consumption.
We here focus on the second step, the equilibrium level of \(k^*\) determines consumption and thus consumption is a function of \(k^*\). At a lower saving rate (see Figure 2), \(k^*\) is too small, so there is less consumption. At a higher saving rate, \(k^*\) is too large, so there is also less consumption. Therefore, we can find that consumption is in a quadratic form w.r.t. \(k^*\).
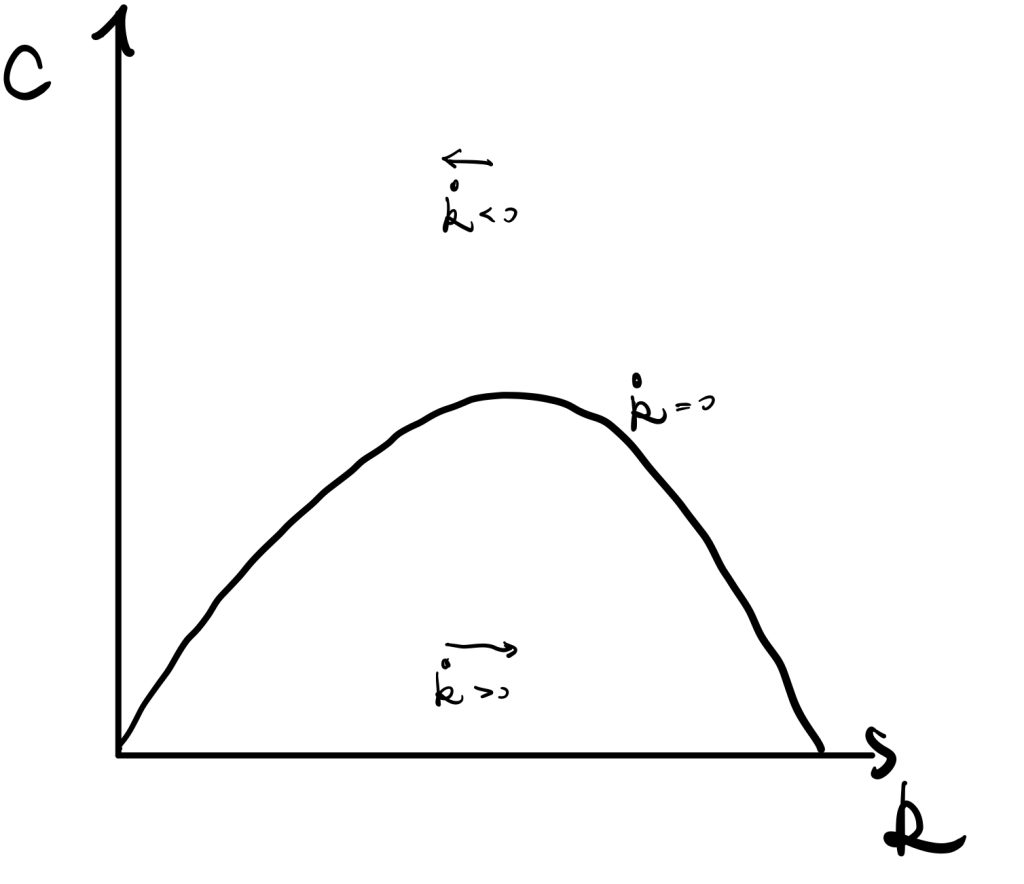
$$ (s\downarrow) \Leftrightarrow k \downarrow \to c \downarrow$$
$$ (s\uparrow) \Leftrightarrow k \uparrow \to c \downarrow$$
$$ (s_{GoldenRule}) \Leftrightarrow k^*\to c_{max}$$
Or, we can consider consumption as the difference between \(y\) and \((n+g)k\). The wedge like area gets large and then shrinks.
Overall, the above facts make the \(\dot{k}=0\) curve.
Recall \( \dot{k}(t)=f(k(t))-c(t)-(n+g)k(t) \).
Above the curve where \(c\) is large, then \(\dot{k}<0\) so \(k\) decreases. Below the curve where \(c\) is small, then \(\dot{k}>0\) so \(k\) increases. Implication is that if less consumption, then more saving, \(\dot{k}\) increases.
Phase Diagram.
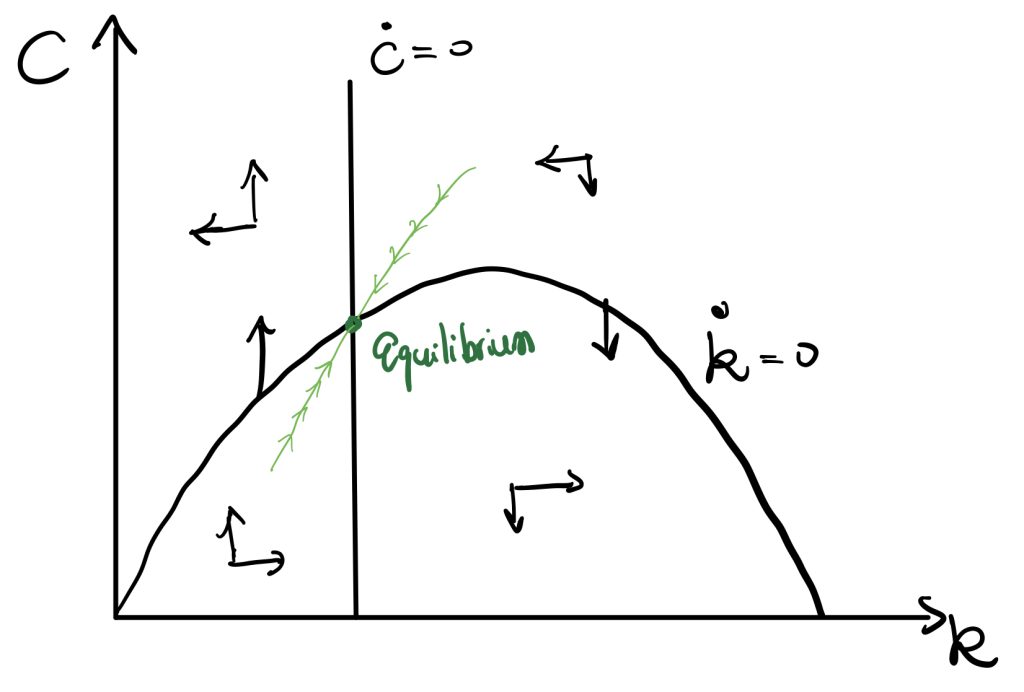
Combining Figure 1 and Figure 3, we get the above Phase Diagram. The equilibrium is shown in the figure above.
P.S. We can prove that the equilibrium is less than Golden Rule level \(k^*_{GoldenRule}\) (which is the maximum point in the quadratic shaped curve). The proof is the following,
The value of k at \(\dot{c}=0\) is \(f'(k)-\rho-\theta g=0\), and the Golden Rule level is \(c=f(k)-(n+g)k\) (as we illustrated before), and take f.o.c. w.r.t. k to solve the Golden rule k. \(\frac{\partial c}{\partial k}=0 \to f'(k)=n+g\). Therefore, we get,
$$ \begin{cases} f_1:=f'(k_{equilibrium})=\rho+\theta g \quad\text{equilibrium in phase diagram}\\ f_2:=f'(k_{GoldenRule})=n+g\quad\text{golden rule level}\end{cases}$$
$$ \rho+\theta g>n+g \quad \text{by our assumption of \beta convergence}$$
So, we get,
$$ f_1>f_2 $$
$$ k_{equilibrium}< k_{GoldenRule} $$
Thus, we find the equilibrium level capital per efficient workers, \(k_{equilibrium}\), must be less than the Golden Rule level \( k_{GoldenRule} \).
From the phase diagram, we can get the saddle path that can achieve equilibrium.
BGP
At the Balanced Growth Path, the economy is in equilibrium. So the time-paths satisfy \( \frac{\dot{c}}{c}=0, and \frac{\dot{k}}{k}=0 \). Therefore, we can get the BGP of others,
$$y=k^{\alpha}\to lny=\alpha ln(k)\to \frac{\dot{y}}{y}= \alpha\frac{\dot{k}}{k}=0 $$
$$y=\frac{Y}{AL}\to lny=lnY-lnA-lnL\to \frac{\dot{y}}{y}=0= \frac{\dot{Y}}{Y}- (g+n)\\ \Rightarrow \frac{\dot{Y}}{Y}= g+n $$
$$ \frac{\dot{c}}{c}=0\to c=\frac{C}{AL} \to \frac{\dot{c}}{c} =g+n $$
Similarly (also as the similar method in Solow model), we can get,
$$ \begin{cases} \frac{\dot{Y}}{Y}=n+g \\ \frac{\dot{C}}{C}=n+g \\ \frac{\dot{K/L}}{K/L}=g \\ \frac{\dot{Y/L}}{Y/L}=g \\ \frac{\dot{C/L}}{C/L}=g \end{cases} $$
Fiscal Policy
Assumption: government conducts government purchases, \(G(t)\). The government purchases do not affect the utility of private sectors, and future outputs. Government finances, G(t), by lump-sum taxes.
We can consider the crowding-out effect. Under full employment, government purchases take away part of the consumptions. In our case, government spending takes away some of the savings. Therefore, the dynamics of capital per efficient workers become (the minus government spending term shifts the curve downward by G(t)),
$$\dot{k}(t)=f(k(t))-c(t)-G(t)-(n+g)k(t)$$
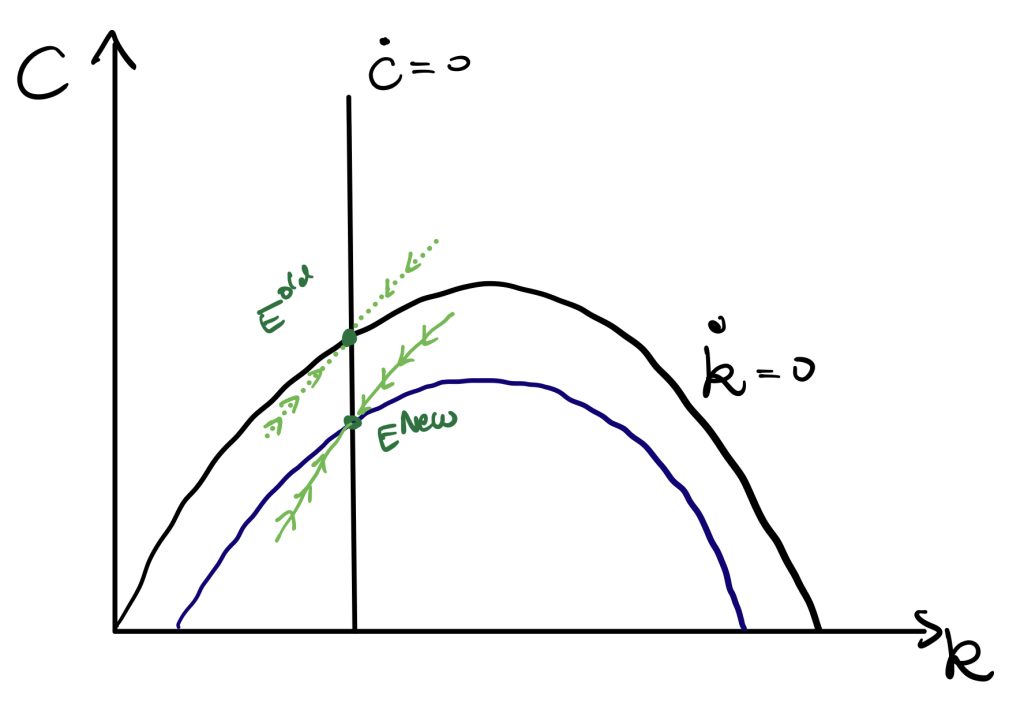
In short, government purchases would make the economy achieve a new equilibrium where there is less consumption but the same capital (investment from the private sector) level. Also, the saddle path moves downward.
\(\rho\) Changes
A fall in \(\rho\) can be considered as the effect of monetary policy. A fall in \(\rho\) would result in a movement of \(\dot{c}=0\) curve to the right by the equality, \(\frac{\dot{c}}{c}= \frac{r(t)-\rho-\theta g}{\theta} =\frac{f'(k)-\rho-\theta g}{\theta}\).
P.S. \(\dot{c}=0 \Leftrightarrow f'(k)=\rho-\theta g \to k=f’^{-1}( \rho-\theta g )\)
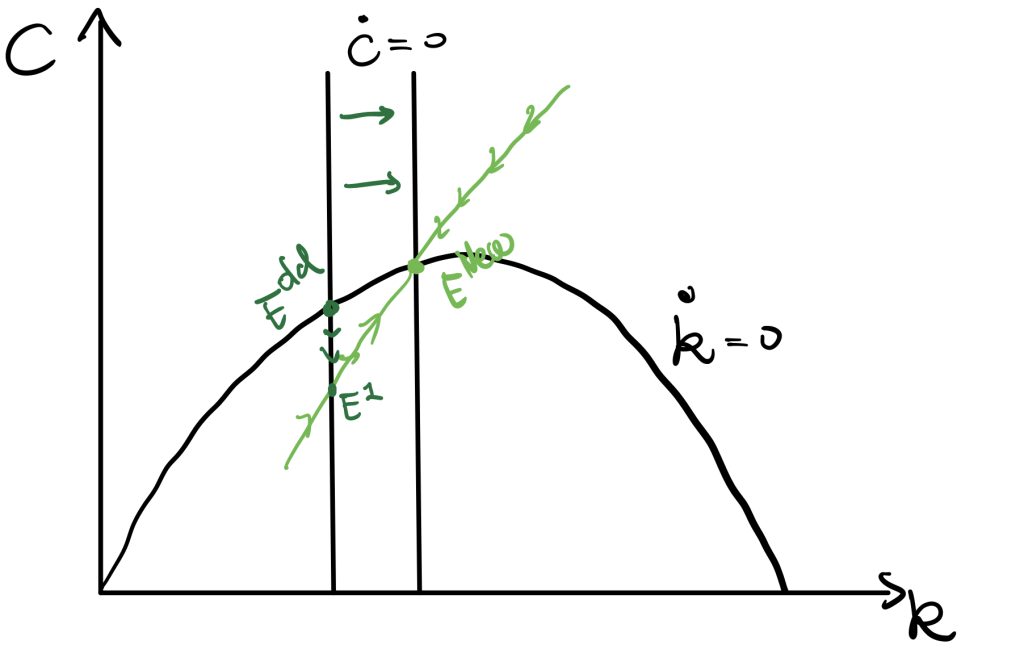
As \(\rho\) changes, a new path generates. The economy is at \(E^1\), and then follows the new path moving to \(E^{new}\). We would finally end up with a new equilibrium with higher consumption.
Convergence
See Romer (2018) find the speed of adjustment.
Recall two paths in the R.C.K model.
$$ \dot{k}=f(k(t))-c(t)-(n+g)k(t) $$
$$ \frac{\dot{c}}{c}=\frac{f'(k)-\rho-\theta g}{\theta} $$
We replace this non-linear equation with the linear approximation, so we take the first order Taylor approximation around the equilibrium \(k^*\) and \(c^*\).
$$ \dot{k} _{approx} \approx \dot{k}|_{k^*,c^*}+\frac{\partial \dot{k}}{\partial k}|_{ k^*,c^* }(k-k^*) \\+ \frac{\partial \dot{k}}{\partial c}|_{ k^*,c^* }(c-c^*) $$
As in the equilibrium \( \dot{k}|_{ k^*,c^* } =0\) and \( \dot{k}|_{ k^*,c^* }=0 \), so
$$ \dot{k}_{approx}\approx \frac{\partial \dot{k}}{\partial k}|_{ k^*,c^* }(k-k^*)+\frac{\partial \dot{k}}{\partial c}|_{ k^*,c^* }(c-c^*) $$
Similarly, (after we doing a bit transformation \( \dot{c}=\frac{f'(k)-\rho-\theta g}{\theta}c \) ).
$$ \dot{c} _{approx} \approx \frac{\partial \dot{c}}{\partial k}|_{ k^*,c^* }(k-k^*)+\frac{\partial \dot{c}}{\partial c}|_{ k^*,c^* }(c-c^*) $$
We then replace \( \dot{c}=\frac{f'(k)-\rho-\theta g}{\theta}c \) ) and \( \dot{k}=f(k(t))-c(t)-(n+g)k(t) \) into \( \dot{k}_{approx} \) and \( \dot{c}_{approx} \). Also, we denote \(\tilde{c}=c-c^*\) and \(\tilde{k}=k-k^*\).
P.S. \(\partial \frac{\dot{c}}{\partial k}|_{ k^*,c^* }=\frac{c^*}{\theta}f”(k^*)\) and \(\frac{\partial \dot{c}}{\partial c}|_{ k^*,c^* }= \frac{f'(k)-\rho-\theta g}{\theta} |_{ k^*,c^* }=\frac{\dot{c}}{c}|_{ k^*,c^* }=0\).
And, \(\frac{\partial \dot{k}}{\partial k}|_{ k^*,c^* }=f'(k^*)-(\delta+n) \) and \(\frac{\partial \dot{k}}{\partial c}|_{ k^*,c^* }=-1\).
Then, we get,
$$ \dot{k}_{approx}\approx [f'(k^*)-(\delta+n)] \tilde{k}-\tilde{c} $$
In equilibrium, \(\dot{c}=0 \Leftrightarrow f'(k)=\rho+\theta g\), so,
$$ \dot{k}_{approx}\approx [\rho+\theta g-(\delta+n)] \tilde{k}-\tilde{c} =\beta \tilde{k}-\tilde{c} $$
$$ \dot{c} _{approx} \approx \frac{c^* f”(k^*)}{\theta } \tilde{k}$$
Then, we divide both side by \(tilde{k}\) or \(\tilde{c}\), respectively. We would get,
$$ \frac{\dot{k}_{approx}}{\tilde{k}}\approx \beta -\frac{\tilde{c}}{\tilde{k}} $$
$$ \frac{\dot{c} _{approx}}{\tilde{c}} \approx \frac{c^* f”(k^*)}{\theta } \frac{\tilde{k}}{\tilde{c}} $$
From the above two equations we can find growth rate of \(\dot{\tilde{c}}_{approx}\) and \( \dot{\tilde{k}}_{approx} \) depend only on the ratio, \(\frac{\tilde{k}}{\tilde{c}}\).
Later, we apply a very strong assumption that \(\tilde{c}\) and \(\tilde{k}\) changes at the same rate, and also the rate make LHS of two equations equal. By this assumption, we denote,
$$ \frac{\dot{c} _{approx}}{\tilde{c}}= \frac{\dot{k}_{approx}}{\tilde{k}} =\mu $$
Then, solving those three equations by making above two equations equal, we would get,
$$\mu^2-\beta \mu +\frac{ f”(k^*) c^* }{\theta}=0$$
$$\mu_1,\mu_2=\frac{\beta\pm[\beta^2-4f”(k^8)c^*/\theta]^{1/2}}{2}$$
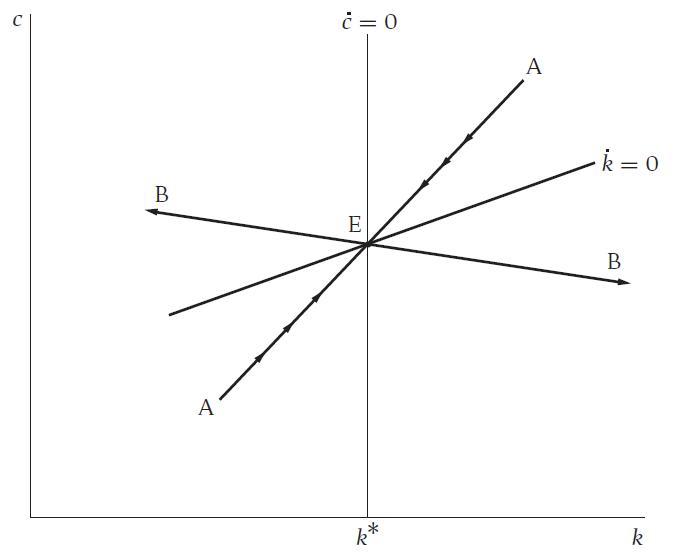
We can see \(\mu\) must be negative, otherwise the economy cannot converge (see the path BB). If \(\mu<0\), the economy would be in the path AA instead. The path is the saddle path of R.C.K. model.
Applying such as the Cobb-Douglas form production, we can plug second derivatives of the production into \(\mu\) and get the speed of adjustment.